Hyaluronic Acid-Decorated Laponite® Nanocomposites for Targeted Anticancer Drug Delivery
Abstract
: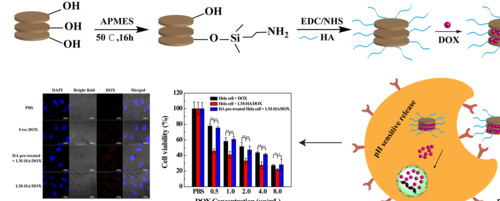
Graphical Abstract
1. Introduction
2. Experiment
2.1. Preparation and Characterization of DOX-Loaded Nanocomposites
2.2. Cell Culture
2.3. In Vitro Cytotoxicity Assay and Cell Morphology Observation
2.4. Cellular Uptake
3. Results and Discussion
3.1. Synthesis and Characterization of LM-HA/DOX
3.2. DOX Encapsulation and Release
3.3. Targeted Therapeutic Efficacy of LM-HA/DOX
3.4. CD44-Mediated Cellular Uptake
4. Conclusions
Supplementary Materials
Author Contributions
Funding
Conflicts of Interest
References
- Liu, D.; Yang, F.; Xiong, F.; Gu, N. The Smart Drug Delivery System and Its Clinical Potential. Theranostics 2016, 6, 1306–1323. [Google Scholar] [CrossRef] [PubMed] [Green Version]
- Oku, N. Innovations in Liposomal DDS Technology and Its Application for the Treatment of Various Diseases. Biol. Pharm. Bull. 2017, 40, 119–127. [Google Scholar] [CrossRef] [PubMed] [Green Version]
- Xu, F.; Liu, M.; Li, X.; Xiong, Z.; Cao, X.; Shi, X.; Guo, R. Loading of indocyanine green within polydopamine-coated laponite nanodisks for targeted cancer photothermal and photodynamic therapy. Nanomaterials 2018, 8, 347. [Google Scholar] [CrossRef] [PubMed]
- Zhu, J.; Zheng, L.; Wen, S.; Tang, Y.; Shen, M.; Zhang, G.; Shi, X. Targeted cancer theranostics using alpha-tocopheryl succinate-conjugated multifunctional dendrimer-entrapped gold nanoparticles. Biomaterials 2014, 35, 7635–7646. [Google Scholar] [CrossRef] [PubMed]
- Wang, D.; Yan, J.; Jiang, J.; Liu, X.; Tian, C.; Xu, J.; Yuan, M.; Han, X.; Wang, J. Polydiacetylene liposomes with phenylboronic acid tags: A fluorescence turn-on sensor for sialic acid detection and cell-surface glycan imaging. Nanoscale 2018, 10, 4570–4578. [Google Scholar] [CrossRef] [PubMed]
- Xu, W.; Qian, J.; Hou, G.; Wang, Y.; Wang, J.; Sun, T.; Ji, L.; Suo, A.; Yao, Y. A dual-targeted hyaluronic acid-gold nanorod platform with triple-stimuli responsiveness for photodynamic/photothermal therapy of breast cancer. Acta Biomater. 2018, 1742–1756. [Google Scholar] [CrossRef] [PubMed]
- Alonso, S. Exploiting the bioengineering versatility of lactobionic acid in targeted nanosystems and biomaterials. J. Controlled Release 2018, 287, 216–234. [Google Scholar] [CrossRef]
- Zhao, L.; Zhu, J.; Cheng, Y.; Xiong, Z.; Tang, Y.; Guo, L.; Shi, X.; Zhao, J. Chlorotoxin-Conjugated Multifunctional Dendrimers Labeled with Radionuclide I-131 for Single Photon Emission Computed Tomography Imaging and Radiotherapy of Gliomas. ACS Appl. Mater. Interfaces 2015, 7, 19798–19808. [Google Scholar] [CrossRef]
- Zhang, Y.; Wu, X.; Hou, C.; Shang, K.; Yang, K.; Tian, Z.; Pei, Z.; Qu, Y.; Pei, Y. Dual-responsive dithio-polydopamine coated porous CeO2 nanorods for targeted and synergistic drug delivery. Int. J. Nanomed. 2018, 13, 2161–2173. [Google Scholar] [CrossRef] [PubMed] [Green Version]
- Zhang, Y.; Guo, Z.; Cao, Z.; Zhou, W.; Zhang, Y.; Chen, Q.; Lu, Y.; Chen, X.; Guo, Q.; Li, C.; et al. Endogenous albumin-mediated delivery of redox-responsive paclitaxel-loaded micelles for targeted cancer therapy. Biomaterials 2018, 183, 243–257. [Google Scholar] [CrossRef]
- Hatakeyama, H. Development of a Novel Liposomal DDS by Manipulating Pharmacokinetics and Intracellular Trafficking for Drug Therapy and Nucleic Acid Medicine. Yakugaku Zasshi 2018, 138, 591–598. [Google Scholar] [CrossRef] [PubMed] [Green Version]
- Wang, Y.; Guo, R.; Cao, X.; Shen, M.; Shi, X. Encapsulation of 2-methoxyestradiol within multifunctional poly (amidoamine) dendrimers for targeted cancer therapy. Biomaterials 2011, 32, 3322–3329. [Google Scholar] [CrossRef] [PubMed]
- Wang, F.; Chen, Y.; Zhang, D.; Zhang, Q.; Zheng, D.; Hao, L.; Liu, Y.; Duan, C.; Jia, L.; Liu, G. Folate-mediated targeted and intracellular delivery of paclitaxel using a novel deoxycholic acid-o-carboxymethylated chitosan-folic acid micelles. Int. J. Nanomed. 2012, 7, 325–337. [Google Scholar]
- Zhou, T.; Xiao, C.; Fan, J.; Chen, S.; Shen, J.; Wu, W.; Zhou, S. A nanogel of on-site tunable pH-response for efficient anticancer drug delivery. Acta Biomater. 2013, 9, 4546–4557. [Google Scholar] [CrossRef] [PubMed]
- Rahoui, N.; Jiang, B.; Hegazy, M.; Taloub, N.; Wang, Y.; Yu, M.; Huang, Y.D. Gold modified polydopamine coated mesoporous silica nano-structures for synergetic chemo-photothermal effect. Colloids Surf. B 2018, 171, 176–185. [Google Scholar] [CrossRef] [PubMed]
- Yu, S.; Li, Q.; Wang, J.; Du, J.; Gao, Y.; Zhang, L.; Chen, L.; Yang, Y.; Liu, X. A targeted drug delivery system based on carbon nanotubes loaded with lobaplatin toward liver cancer cells. J. Mater. Res. 2018, 33, 2565–2575. [Google Scholar] [CrossRef]
- Ingram, S.E.; Liggat, J.J.; Pethrick, R.A. Properties of epoxy nanoclay system based on diaminodiphenyl sulfone and diglycidyl ether of bisphenol F: Influence of post cure and structure of amine and epoxy. Polym. Int. 2007, 56, 1029–1034. [Google Scholar] [CrossRef]
- Ding, L.; Hu, Y.; Luo, Y.; Zhu, J.; Wu, Y.; Cao, X.; Peng, C.; Shi, X.; Guo, R. LAPONITEreg-stabilized iron oxide nanoparticles for in vivo MR imaging of tumors. Biomater. Sci. 2016, 4, 474–482. [Google Scholar] [CrossRef]
- Li, Y.; Maciel, D.; Tomás, H.; Rodrigues, J.; Ma, H.; Shi, X. pH sensitive laponite/alginate hybrid hydrogels: Swelling behaviour and release mechanism. Soft Matter 2011, 7, 6231–6238. [Google Scholar] [CrossRef]
- Wang, S.; Wu, Y.; Guo, R.; Huang, Y.; Wen, S.; Shen, M.; Wang, J.; Shi, X. Laponite nanodisks as an efficient platform for doxorubicin delivery to cancer cells. Langmuir 2013, 29, 5030–5036. [Google Scholar] [CrossRef]
- Zhuang, Y.; Zhou, L.; Zheng, L.; Hu, Y.; Ding, L.; Li, X.; Liu, C.; Zhao, J.; Shi, X.; Guo, R. Laponite-polyethylenimine based theranostic nanoplatform for tumor-targeting CT imaging and chemotherapy. ACS Biomater. Sci. Eng. 2017, 3, 431–442. [Google Scholar] [CrossRef]
- Wang, S.; Zheng, F.; Huang, Y.; Fang, Y.; Shen, M.; Zhu, M.; Shi, X. Encapsulation of amoxicillin within laponite-doped poly (lactic-co-glycolic acid) nanofibers: Preparation, characterization, and antibacterial activity. ACS Appl. Mater. Interfaces 2012, 4, 6393–6401. [Google Scholar] [CrossRef] [PubMed]
- Ghadiri, M.; Hau, H.; Chrzanowski, W.; Agus, H.; Rohanizadeh, R. Laponite clay as a carrier for in situ delivery of tetracycline. RSC Adv. 2013, 3, 20193–20201. [Google Scholar] [CrossRef]
- Fraile, J.M.; Garcia-Martin, E.; Gil, C.; Mayoral, J.A.; Pablo, L.E.; Polo, V.; Prieto, E.; Vispe, E. Laponite as carrier for controlled in vitro delivery of dexamethasone in vitreous humor models. Eur. J. Pharm. Biopharm. 2016, 108, 83–90. [Google Scholar] [CrossRef] [PubMed] [Green Version]
- Wu, Y.; Guo, R.; Wen, S.; Shen, M.; Zhu, M.; Wang, J.; Shi, X. Folic acid-modified laponite nanodisks for targeted anticancer drug delivery. J. Mater. Chem. B 2014, 2, 7410–7418. [Google Scholar] [CrossRef]
- Chen, G.; Li, D.; Li, J.; Cao, X.; Wang, J.; Shi, X.; Guo, R. Targeted doxorubicin delivery to hepatocarcinoma cells by lactobionic acid-modified laponite nanodisks. New J. Chem. 2015, 39, 2847–2855. [Google Scholar] [CrossRef]
- Lim, E.K.; Kim, H.O.; Jang, E.; Park, J.; Lee, K.; Suh, J.S.; Huh, Y.M.; Haam, S. Hyaluronan-modified magnetic nanoclusters for detection of CD44-overexpressing breast cancer by MR imaging. Biomaterials 2011, 32, 7941–7950. [Google Scholar] [CrossRef]
- Lee, H.; Park, H.; Noh, G.J.; Lee, E.S. pH-responsive hyaluronate-anchored extracellular vesicles to promote tumor-targeted drug delivery. Carbohydr. Polym. 2018, 202, 323–333. [Google Scholar] [CrossRef]
- Jang, E.; Lim, E.K.; Choi, Y.; Kim, E.; Kim, H.O.; Kim, D.J.; Suh, J.S.; Huh, Y.M.; Haam, S. pi-Hyaluronan nanocarriers for CD44-targeted and pH-boosted aromatic drug delivery. J. Mater. Chem. B 2013, 1, 5686–5693. [Google Scholar] [CrossRef]
- Mandaliti, W.; Nepravishta, R.; Pica, F.; Vallebona, P.S.; Garaci, E.; Paci, M. Thymosin 1 interacts with hyaluronic acid electrostatically by its terminal sequence lkekk. Molecules 2017, 22, 1843. [Google Scholar] [CrossRef]
- Mo, Y.; Wang, H.; Liu, J.; Lan, Y.; Guo, R.; Zhang, Y.; Xue, W.; Zhang, Y. Controlled release and targeted delivery to cancer cells of doxorubicin from polysaccharide-functionalised single-walled carbon nanotubes. J. Mater. Chem. B 2015, 3, 1846–1855. [Google Scholar] [CrossRef]
- He, X.; Liu, B.; Xu, C.; Zhuo, R.; Cheng, S. A multi-functional macrophage and tumor targeting gene delivery system for the regulation of macrophage polarity and reversal of cancer immunoresistance. Nanoscale 2018, 10, 15578–15587. [Google Scholar] [CrossRef] [PubMed]
- Cheng, C.; Meng, Y.; Zhang, Z.; Li, Y.; Zhang, Q. Tumoral acidic pH-responsive cis-diaminodichloroplatinum-incorporated Cy5.5-PEG-g-A-HA nanoparticles for targeting delivery of cddp against cervical cancer. ACS Appl. Mater. Interfaces 2018, 10, 26882–26892. [Google Scholar] [CrossRef] [PubMed]
- Mattheolabakis, G.; Milane, L.; Singh, A.; Amiji, M.M. Hyaluronic acid targeting of CD44 for cancer therapy: From receptor biology to nanomedicine. J. Drug Targeting 2015, 23, 605–618. [Google Scholar] [CrossRef] [PubMed]
- Parafiniuk, K.; Monnereau, C.; Sznitko, L.; Mettra, B.; Zelechowska, M.; Andraud, C.; Miniewicz, A.; Mysliwiec, J. Distributed feedback lasing in amorphous. Polymers with covalently bonded fluorescent dyes: The influence of photoisomerization process. Macromolecules 2017, 50, 6164–6173. [Google Scholar] [CrossRef]
- Mayol, L.; de Stefano, D.; de Falco, F.; Carnuccio, R.; Maiuri, M.C.; de Rosa, G. Effect of hyaluronic acid on the thermogelation and biocompatibility of its blends with methyl cellulose. Carbohydr. Polym. 2014, 112, 480–485. [Google Scholar] [CrossRef]
- Hang, C.; Zou, Y.; Zhong, Y.; Zhong, Z.; Meng, F. NIR and UV-responsive degradable hyaluronic acid nanogels for CD44-targeted and remotely triggered intracellular doxorubicin delivery. Colloids Surf. B 2017, 158, 547–555. [Google Scholar] [CrossRef]
- Daehler, A.; Boskovic, S.; Gee, M.L.; Separovic, F.; Stevens, G.W.; O’Connor, A.J. Postsynthesis vapor-phase functionalization of MCM-48 with hexamethyldisilazane and 3-aminopropyldimethylethoxylsilane for bioseparation applications. J. Phys. Chem. B 2005, 109, 16263–16271. [Google Scholar] [CrossRef]
- Sun, F.; Yu, Y.; Yang, Z.; Wang, Z.; Li, Y.; Wang, F.; Tan, H. Hyaluronic acid-endostatin2-alft1 (HA-ES2-AF) nanoparticle-like conjugate for the target treatment of diseases. J. Controlled Release 2018, 288, 1–13. [Google Scholar] [CrossRef]
- Chahuki, F.F.; Aminzadeh, S.; Jafarian, V.; Tabandeh, F.; Khodabandeh, M. Hyaluronic acid production enhancement via genetically modification and culture medium optimization in Lactobacillus acidophilus. Int. J. Biol. Macromol. 2019, 121, 870–881. [Google Scholar] [CrossRef]
- Zhang, B.; Chang, B.; Sun, T. Synthesis and Study of Hypoxia-Responsive Micelles Based on Hyaluronic Acid. Acta Chim. Sin. 2018, 76, 35–42. [Google Scholar] [CrossRef]
- Luo, Z.; Li, Y.; Wang, B.; Jiang, J. pH-Sensitive Vesicles Formed by Amphiphilic Grafted Copolymers with Tunable Membrane Permeability for Drug Loading/Release: A Multiscale Simulation Study. Macromolecules 2016, 49, 6084–6094. [Google Scholar] [CrossRef]
Materials | ζ-Potential (mV) | Hydrodynamic Size (nm) |
---|---|---|
LAP | −39.6 ± 0.7 | 86.5 ± 5.1 |
LM-NH2 | −12.0 ± 2.0 | 184.0 ± 1.9 |
LM-HA | −14.1 ± 1.4 | 282.6 ± 12.7 |
LM-HA/DOX | −10.8 ± 0.7 | 412.7 ± 16.1 |
© 2019 by the authors. Licensee MDPI, Basel, Switzerland. This article is an open access article distributed under the terms and conditions of the Creative Commons Attribution (CC BY) license (http://creativecommons.org/licenses/by/4.0/).
Share and Cite
Jiang, T.; Chen, G.; Shi, X.; Guo, R. Hyaluronic Acid-Decorated Laponite® Nanocomposites for Targeted Anticancer Drug Delivery. Polymers 2019, 11, 137. https://doi.org/10.3390/polym11010137
Jiang T, Chen G, Shi X, Guo R. Hyaluronic Acid-Decorated Laponite® Nanocomposites for Targeted Anticancer Drug Delivery. Polymers. 2019; 11(1):137. https://doi.org/10.3390/polym11010137
Chicago/Turabian StyleJiang, Tingting, Guangxiang Chen, Xiangyang Shi, and Rui Guo. 2019. "Hyaluronic Acid-Decorated Laponite® Nanocomposites for Targeted Anticancer Drug Delivery" Polymers 11, no. 1: 137. https://doi.org/10.3390/polym11010137